ACE2 and SARS-CoV2
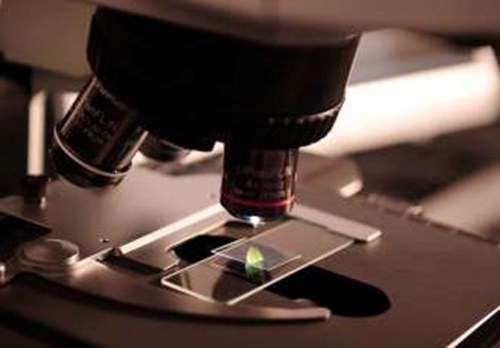
In the winter of 2019, Chinese authorities reported a number of human pneumonia cases in Wuhan City, China. This disease, which was termed as Coronavirus disease 2019 (COVID-19), showed symptoms such as fever and dyspnea, as well as a variation of other symptoms. Whole-genome sequencing results showed that the causative agent was a novel coronavirus, now known as SARS-CoV-2 (1). This initiated a global response in research towards increasing our understanding of the physiology and pathology of the virus.
A key molecule for viral entry of SARS-CoV-2 is the metalloprotease angiotensin-converting enzyme 2 (ACE2), a transmembrane protein consisting of an N-terminal extracellular domain and a C-terminal membrane anchor domain, and encoded by the human Ace2 gene (2). The primary physiological role of ACE2 is the maturation of angiotensin (Ang), which is a hormone responsible for the regulation of vasoconstriction and blood pressure (3).
Using scRNAseq and RNA in situ hybridisation, ACE2 expression in the airways has been observed to be relatively high in nasal epithelium and progressively lower in the bronchial and alveolar regions. Highest expression is seen in goblet and ciliated cells of the nasal epithelium, and ACE2 protein localises to the membrane of motile cilia of respiratory tract epithelia. Airway multiciliated cells appear to be one of the main targets of SARS-CoV-2 infection, suggesting that cilia are also important in SARS-CoV-2 infection, possibly because their extension from the cell surface makes ACE2 more accessible to the virus. Importantly, ACE2 expression correlates with levels of infection of SARS-CoV-2 isolates from patients in different airway compartments. These studies have established the upper airway as the main site of SARS-CoV-2 infection (4).
It has been shown that ACE2 plays an important role in protection from acute lung injury. Ace2 expression is downregulated in mice models of acute lung injury and Ace2 knockout mice show a more severe acute lung injury phenotype. Additionally, it was observed in pig models of lung injury in which ACE2 is overexpressed (5). Although the molecular mechanism by which ACE2 protects against acute lung injury remains unclear, it is known that the carboxypeptidase function of ACE2 is required to confer this protection and that AT2 (AngII receptor 2) also confers protection (2).
Viral entry of SARS-COV-2 into cells is facilitated via the spike (S) protein of coronaviruses, where the surface unit, S1, binds to cellular receptors, such as ACE2 (4). Additionally, entry of SARS-Cov-2 into cells occurs through S protein cleavage by cellular proteases, where S protein is cleaved into S1 and S2 subunits. The S1 subunit contains the receptor binding domain (RBD) which directly binds to the peptidase domain of ACE2, while S2 is involved with membrane fusion (6). Computational analysis of these interactions between the RBD of the SARS-Cov-2 S1 subunit and ACE2 have suggested that SARS-Cov-2 may bind more efficiently to human ACE2 than SARS-CoV (7).
Upon engagement of ACE2 by a receptor binding domain (RBD) in S1, conformational rearrangements occur that cause S1 shedding, cleavage of S2 by host proteases, and exposure of a fusion peptide adjacent to the S2' proteolysis site. Folding of S to a post-fusion conformation is coupled to host cell–virus membrane fusion and cytosolic release of viral RNA (8).
In the case of ACE2, a key entry activator is the protease TMPRSS2. The extracellular domain of ACE2 is cleaved by the TMPRSS2, resulting in the internalisation of the transmembrane domain of ACE2 within the virus (9). The co-expression of ACE2 and TMPRSS2 expressions are widely distributed across human tissues including lung, liver, kidney, heart, brain and multiple digestive tract organs. Additionally, it has been recorded that patients suffering with severe COVID-19 were more likely to have acute cardiac injury, acute kidney injury, liver injury, gastrointestinal injury or nervous system injury (10) Further study of ACE2 and its interactions with proteases such as TMPRSS2 may lead to new avenues for therapeutic and vaccine research (11).
Previous work on emerging viruses, such as SARS-CoV and MERS-CoV, have shown the importance and usefulness of using cell culture models to study and characterise viral replication kinetics, cell tropism, and viral damage profiles of novel and emerging viruses (12). Due mainly to the SARS-CoV outbreak in the early 2000s, several cell lines have been well characterised for their expression of ACE2, and subsequently, their use in coronavirus research. Studies have shown that lytic replication of SARS-CoV occurs in Vero, Vero E6, and MA104, and various other green monkey kidney epithelial cell lines. Additionally, non-lytic replication occurs in CaCo-2 (human colon epithelial), Mv 1 Lu (Aleutian mink lung epithelial), and PK (porcine kidney epithelial) cell lines (5).
Many of these cell lines are available at ECACC. These cell lines can be used to develop new assays which may offer up new pharmaceutical and vaccine options. In vitro models can provide a solid grounding for research, and may be of particular benefit due to the multi-systemic pathogenesis of the disease. It is thanks to this type of work that our understanding of this virus has come far in such a short period of time, and may offer up new insights and considerations in the future.
References
1. Wang Q, Zhang Y, Wu L, Niu S, Song C, Zhang Z, et al. Structural and Functional Basis of SARS-CoV-2 Entry by Using Human ACE2. Cell. 2020;181(4):894-904.e9.
2. Tipnis SR, Hooper NM, Hyde R, Karran E, Christie G, Turner AJ. A Human Homolog of Angiotensin-converting Enzyme. Journal of Biological Chemistry. 2000;275(43):33238-43.
3. Donoghue M, Hsieh F, Baronas E, Godbout K, Gosselin M, Stagliano N, et al. A Novel Angiotensin-Converting Enzyme–Related Carboxypeptidase (ACE2) Converts Angiotensin I to Angiotensin 1-9. Circulation Research. 2000;87(5):e1-e9.
4. Shang J, Wan Y, Luo C, Ye G, Geng Q, Auerbach A, et al. Cell entry mechanisms of SARS-CoV-2. Proceedings of the National Academy of Sciences. 2020;117(21):11727-34.
5. Mossel EC, Huang C, Narayanan K, Makino S, Tesh RB, Peters CJJov. Exogenous ACE2 expression allows refractory cell lines to support severe acute respiratory syndrome coronavirus replication. 2005;79(6):3846-50.
6. Yan R, Zhang Y, Li Y, Xia L, Guo Y, Zhou Q. Structural basis for the recognition of SARS-CoV-2 by full-length human ACE2. Science. 2020;367(6485):1444-8.
7. Wan Y, Shang J, Graham R, Baric RS, Li F. Receptor Recognition by the Novel Coronavirus from Wuhan: an Analysis Based on Decade-Long Structural Studies of SARS Coronavirus. Journal of Virology. 2020;94(7).
8. Chan KK, Dorosky D, Sharma P, Abbasi SA, Dye JM, Kranz DM, et al. Engineering human ACE2 to optimize binding to the spike protein of SARS coronavirus 2. Science. 2020;369(6508):1261-5.
9. Hoffmann M, Kleine-Weber H, Schroeder S, Krüger N, Herrler T, Erichsen S, et al. SARS-CoV-2 Cell Entry Depends on ACE2 and TMPRSS2 and Is Blocked by a Clinically Proven Protease Inhibitor. Cell. 2020;181(2):271-80.e8.
10. Martines RB, Ritter JM, Matkovic E, Gary J, Bollweg BC, Bullock H, et al. Pathology and Pathogenesis of SARS-CoV-2 Associated with Fatal Coronavirus Disease, United States. Emerging Infectious Diseases. 2020;26(9):2005-15.
11. Dong M, Zhang J, Ma X, Tan J, Chen L, Liu S, et al. ACE2, TMPRSS2 distribution and extrapulmonary organ injury in patients with COVID-19. 2020:110678.
12. Chan JF-W, Chan K-H, Choi GK-Y, To KK-W, Tse H, Cai J-P, et al. Differential Cell Line Susceptibility to the Emerging Novel Human Betacoronavirus 2c EMC/2012: Implications for Disease Pathogenesis and Clinical Manifestation. The Journal of Infectious Diseases. 2013;207(11):1743-52.
Written by Gwyain Jones January 2021